Thank you for visiting nature.com. You are using a browser version with limited support for CSS. To obtain the best experience, we recommend you use a more up to date browser (or turn off compatibility mode in Internet Explorer). In the meantime, to ensure continued support, we are displaying the site without styles and JavaScript.
Scientific Reports volume 13, Article number: 10766 (2023 ) Cite this article Penicillamine
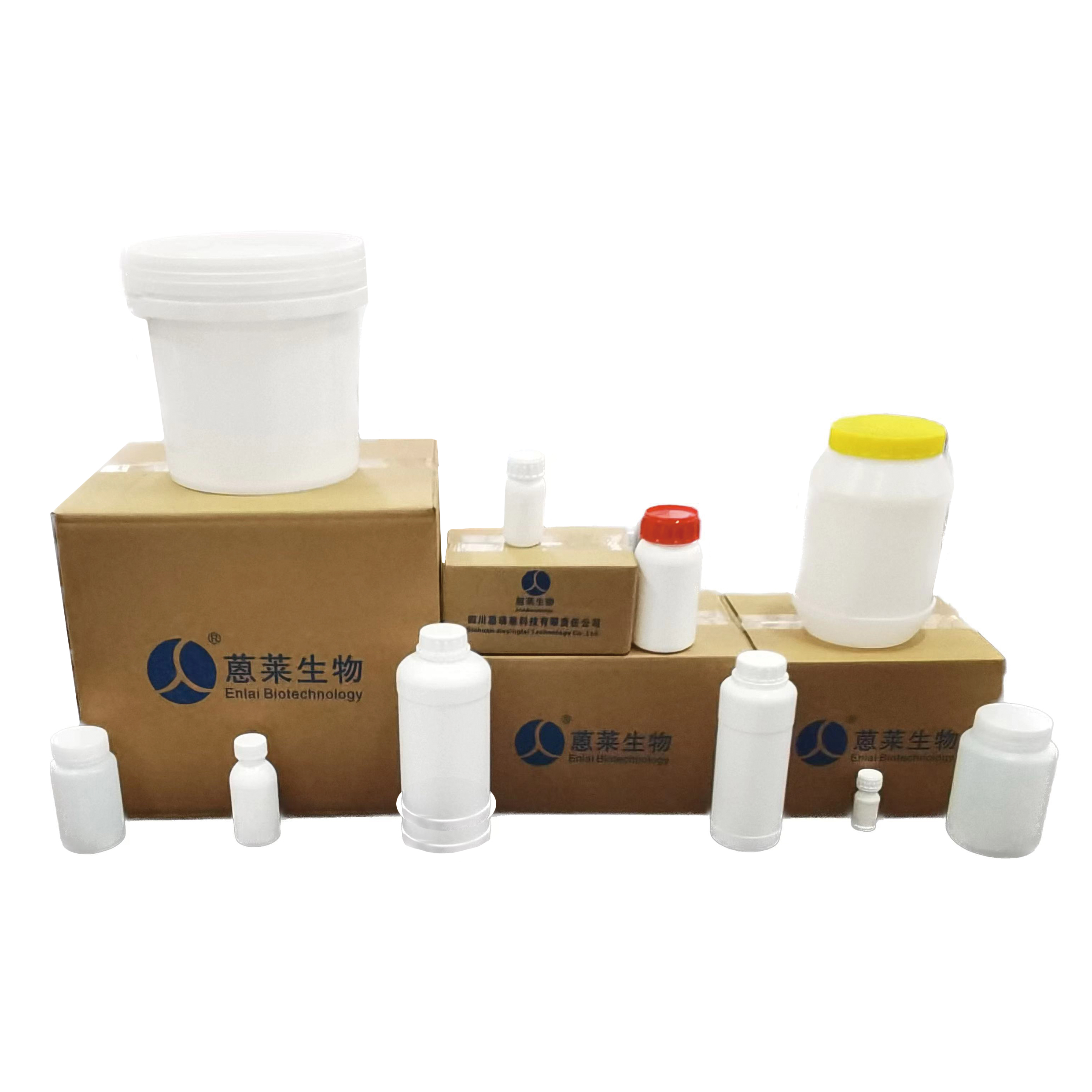
Microbial production of esters has recently garnered wide attention, but the current production metrics are low. Evidently, the ester precursors (organic acids and alcohols) can be accumulated at higher titers by microbes like Escherichia coli. Hence, we hypothesized that their ‘direct esterification’ using esterases will be efficient. We engineered esterases from various microorganisms into E. coli, along with overexpression of ethanol and lactate pathway genes. High cell density fermentation exhibited the strains possessing esterase-A (SSL76) and carbohydrate esterase (SSL74) as the potent candidates. Fed-batch fermentation at pH 7 resulted in 80 mg/L of ethyl acetate and 10 mg/L of ethyl lactate accumulation by SSL76. At pH 6, the total ester titer improved by 2.5-fold, with SSL76 producing 225 mg/L of ethyl acetate, and 18.2 mg/L of ethyl lactate, the highest reported titer in E. coli. To our knowledge, this is the first successful demonstration of short-chain ester production by engineering ‘esterases’ in E. coli.
With increasing global efforts towards sustainability, esters, being biodegradable, are gaining significant recognition for an array of industrial applications, with a major market share in the cosmetics industry, followed by food, lubricants, pharmaceutical, and coating industries1,2. The US esters market, which was 3.8 billion USD in 2019, is estimated to reach 5 billion USD by 2025, primarily driven by increasing demand for emulsifiers and stabilizers in personal care and detergent sectors3. Predominantly, short-chain esters such as ethyl lactate and ethyl acetate occupy the commercial market owing to their biodegradable and environment-friendly characteristics1.
Esters are traditionally synthesized from carboxylic acids and alcohols in the presence of an acid catalyst, typically concentrated sulfuric acid4. However, due to the use of excess alcohol and corrosive chemicals, advanced eco-friendly approaches, like microbial production route, are required to avoid further environmental damage. Microbial cell factories offer a sustainable platform for producing industrial solvents from renewable resources5,6,7,8,9. Biocatalytic esterification can provide an eco-friendly alternative, as it occurs at ambient temperature and does not involve any corrosive waste products. Hence, biomanufacturing of green solvents, like esters, could be an effective substitute10.
Although microbes have long been exploited as cell factories for the production of various chemicals, the catalytic activity of the enzyme/s involved with the engineered pathway/s remains as one of the major bottlenecks. Hence, mining for new enzymes with better activity becomes essential. Under the current investigation, rigorous bioprospecting was performed to find potent esterifying enzymes from various natural producers1,11,12, and Escherichia coli was engineered for the ester production.
Naturally, ester biosynthesis can be accomplished through either of the four categories of enzymes: acyltransferases; esterases; hemiacetal dehydrogenases; and monoxygenases13. All of the earlier reports on microbial ester production have predominantly overexpressed ‘acyltransferase’ enzymes such as alcohol O-acetyltransferase 1 (Atf1) from yeasts, vesca alcohol acyltransferase (VAAT) from the wild strawberry plant (Fig. 1a)1,2,14,15. They require acyl-CoA and alcohol as their substrates, wherein relatively less substrate (acyl-CoA) availability could be one of the primary limitations in acyltransferase catalysis. On the other hand, substrates for ‘esterase’, organic acids and alcohols, are usually accumulated by the microbial hosts like E. coli at relatively higher titers (Fig. 1a)16. Hence, their direct esterification will be favorable. In addition, a further increase in the precursor titers through metabolic engineering can improve the carbon flux towards the ester production17,18. Despite the above advantages, esterases have never been exploited as candidates for ester production via metabolic engineering. A positive Gibbs free energy for biological esterification has been attributed to this limited interest1,2. However, there are pieces of evidence for biological esterification by esterases, which contradict these estimations19,20,21. The Gibbs free energy of reaction estimated in these earlier reports were based on an online interface, eQuilibrator1,2,13. The eQuilibrator uses a default pH and ionic strength of 7.0 and 0.1 M, respectively, to obtain Gibbs free energy at biological conditions (ΔrG′). However, cytoplasmic pH and ionic strength for microorganisms vary widely from this default value22,23,24, and this can introduce error in the Gibbs free energy prediction25. In addition, as these estimations involve mathematical models, like the ‘group contribution method’ that have an average error of 9–10 kJ/mol, we were not convinced with the positive ΔrG′ estimated with this approach26,27. With a wide range of precursor concentrations (Q, reaction quotient) in consideration, ΔrG estimated from ΔrGo (Gibbs free energy of a reaction at standard conditions) for the esterase catalyzed esterification; turned out to be negative, further adding to the conundrum (Fig. 1b). Therefore, experimental validation of the esterase catalyzed esterification route is important owing to the several advantages for rapid progress in ester synthesis. Comprehending this, under the current study, we have engineered E. coli by cloning various esterases, and have analyzed their in vivo esterification potential.
Schematic representation of the metabolic pathways for ester production and in silico ΔrG predicted for ester synthesis via esterification: (a) Ester bioproduction can be achieved via acyltransferase (using acyl-CoA and alcohol as precursors) or esterase (using organic acid and alcohol as precursors) catalysis. The metabolic pathway for engineering E. coli cells for the biosynthesis of short-chain esters (ethyl lactate and ethyl acetate) and precursors thereof, including ethanol, D-lactate, and L-lactate. The thickness of the arrows depicts the average flux in pathway63, dotted arrows indicate the esterification reaction through acyltransferase (AAT) or esterase (Est) routes. Heterologous enzymes (LDH, lactate dehydrogenase; ADH, alcohol dehydrogenase; PCT, propionyl CoA synthase; PDC, pyruvate decarboxylase) that have been overexpressed are represented in red color and bold, whereas the genes encoding for AAT, Est and lactate dehydrogenase have been enlisted in a green, orange, and blue box respectively next to the pathway. (b) Gibbs free energy (ΔrG and ΔrG′) estimation for varying reaction quotients (Q) at different temperatures (30 °C and 37 °C) for the production of ethyl acetate via an esterification reaction. ΔrG°, standard Gibbs free energy of a reaction; and ΔrG′°, standard Gibbs free energy of a reaction at pH 7 and ionic strength of 0.1 M. The image was created using Microsoft PowerPoint and Microsoft Excel.
The current work encompasses in silico mining of various esterases from Brettanomyces bruxellensis AWRI1499, Saccharomyces cerevisiae, Pichia pastoris, and Pseudomonas aeruginosa. The nucleotide sequences for the esterases identified from B. bruxellensis have incorrect non-nucleotide/non-amino acid inclusions in the online databases (NCBI Genbank and Uniprot) and hence, preliminary manual curation was performed based on their sequence homologies. Comparative biochemical analysis of all the enzymes unveiled that esterases could serve as potent esterifying biocatalysts. Furthermore, high cell density (HCD) fermentation and optimization of cultivation conditions showed significant production of ethyl lactate and ethyl acetate from the engineered cells at the laboratory as well as bioreactor scales. Therefore, our research is the first successful demonstration of using esterases to produce short-chain esters: ethyl lactate and ethyl acetate.
Bioprospecting for esterifying enzymes was performed to obtain their genetic sequences from the following natural producers: B. bruxellensis AWRI1499; S. cerevisiae; Pichia pastoris; and Pseudomonas aeruginosa. As a first step, B. bruxellensis proteome was analyzed, and two esterases, acetylxylan-esterase (AXE2) and carbohydrate-esterase (CE) were identified for exploring their esterification potential28,29. Gene/polypeptide sequences for the selected enzymes from B. bruxellensis had incorrect nucleotide/amino acid inclusions in GenBank/ UniProt. Hence, manual curation was performed based on sequence similarities using blastn, blastp, and clustal omega tools (Supplementary Fig. SI-1a). Local alignment (BLAST) and multiple sequence alignment (ClustalO) of the GenBank/UniProt sequences were performed to obtain the most closely related sequences, and the incorrect inclusions were corrected by replacement with the nucleotide/amino acids from the homologous sequence/s (Supplementary Fig. SI-1a). During replacement, the polarity and the hydrophobicity index of the neighboring amino acids were considered. Final nucleotide sequences were subjected to in silico translation (TRANSLATE tool) and analyzed for their folding using the SWISS-MODEL tool. Similarly, model fermentative yeasts Pichia pastoris and S. cerevisiae were explored for their inherent esterifying enzymes, acyl-coenzymeA: ethanol O-acyltransferase (AcAlT) and ethyl-ester-synthase-1 (EEB1), respectively.
Like yeasts, pathogenic bacteria like Pseudomonas are also known to synthesize potent esterases30,31. Interestingly, Esterase-A32 is a membrane bound esterase from Pseudomonas with its inherent signal peptide, and it is completely annotated in protein repositories, including UniProt. Hence, EstA from Pseudomonas aeruginosa was also selected for studying its esterification potential30 (Fig. 1a). Further, to increase its soluble cytoplasmic fraction, we truncated EstA by removing the signal sequence to produce tEstA33,34 (Supplementary Fig. SI-1b). SDS-PAGE results of the soluble fraction showed a more prominent protein band corresponding to tEstA (~ 66 kDa) than EstA indicating successful solubilization (Supplementary Fig. SI-1b). In total, four different esterases (EEB1, EstA, CE, and AXE2), along with an edited tEstA, and a representative acyltransferase (AcAlT) were considered for heterologous cloning into E. coli35,36 (Fig. 1a).
All the genes coding for esterases and an acyltransferase were codon optimized, synthesized, and successfully cloned under the control of T7 promoter (PT7). Phase I E. coli strains were constructed by transforming E. coli BL21-DE3 with the above plasmid constructs (Supplementary Fig. SI-2). The whole cell lysates of the induced Phase I strains were analyzed for ester hydrolysis activity based on pNPA colorimetric assay. kcat/KM (catalytic efficiency) and KM values revealed that EEB1 exhibited the highest catalytic efficiency for pNPA hydrolysis over other candidates (Fig. 2). AcAlT, despite being acyltransferase, showed the second highest efficiency and relatively lower KM (higher affinity for the substrate), presumably due to its broader substrate specificity (Fig. 2). CE and tEstA, on the other hand, indicated relatively moderate hydrolysis activity with higher KM values than that of AcAlT (Fig. 2). However, AXE2 exhibited lower catalytic efficiency, but higher KM comparable that of the BL21-pET lysate control. Therefore, all the enzymes were further investigated for their in vivo esterification potential.
In vitro esterase biocatalysis: (a) In vitro esterase assay using artificial substrate p-nitrophenyl acetate (pNPA) encompasses AAT or Est catalyzed substrate hydrolysis to p-nitrophenol (pNP, yellow colored solution), which can be spectrophotometrically quantified. (b) Comparative pNPA hydrolysis assay using different crude E. coli lysates possessing AAT (AcAlT), esterases (EEB1, tEstA, CE, AXE2), and control (with pET15b). Results indicated a mutually reverse trend of KM and reaction specificity (kcat/KM). The image was created using Microsoft PowerPoint and Microsoft Excel.
As a next step to assess the esterification potential of these heterologous enzymes, the Phase I strains were investigated for the production of ethyl lactate. This part of the study leveraged on the inherent ability of E. coli to produce both ethanol and lactate. BL21-pET strain possessing empty plasmid was used as a negative control. Therefore, first, the control strain BL21-pET possessing empty plasmid (Table 1), was explored for the production of lactate and ethanol through high-cell density (OD600 = 3.0, HCD3) fermentation. Results indicated that the strain could synthesize lactate (3.8 g/L) and ethanol (0.7 g/L) as expected (Supplementary Fig. SI-3a). However, as the ethanol concentration was relatively low, preliminary screening of all the Phase I strains was performed with HCD3, with an external doping of 10 g/L ethanol. However, the ethyl lactate titers amongst the Phase I strains were very less (< 0.1 mg/L) indicating that sole external supplementation of the precursors may not be sufficient (Supplementary Fig. SI-3b). Therefore, the Phase I strains were engineered with the pathways for overproduction of ethanol and lactate, forming Phase II strains (Supplementary Fig. SI-2). The Phase II strains had overexpression of the genes for stereospecific lactate (gldA101 for D-lactate or lldD for L-lactate), and ethanol (pdc and adh genes from Zymomonas mobilis) production (Table 1).
Fermentation studies were conducted with the Phase II strains, to screen for ester production. The preliminary fermentation (OD600 = 3.0, HCD3) results indicated that Phase II strains produced significantly higher ester titers than Phase I strains, which could be attributed to the cloned ethanol and lactate over-production pathways (Fig. 3, Supplementary Fig. SI-3b). It was also observed that ethyl acetate was synthesized at higher titers across all the strains in comparison to ethyl lactate. For instance, SSL77 gave the highest ester titers with 15.8 mg/L of ethyl acetate and 3.4 mg/L of ethyl lactate. In addition, the strains expressing esterases EEB1 (SSL66, SSL67), AXE2 (SSL72, SSL73), CE (SSL74, SSL75), and EstA (SSL76, SSL77), exhibited higher ester titers in comparison to the strain expressing the acyltransferase AcAlT (SSL64, SSL65) (Supplementary Fig. SI-4a; Fig. 3a).
Ester production using high cell density fermentation: (a) Preliminary analysis using high cell density HCD3 has been represented as a comparative plot with concentrations of esters and precursors thereof. Corresponding constructs with D- or L-lactate dehydrogenases have been enlisted. The experiment was performed with intermittent aliquot sampling. (b) Ethyl lactate production was analyzed at HCD10 with sample-wise cultivation method, where every sample variant and the replicate was cultivated in a separate tube to avoid any oxygen interference during sampling. E. coli strains possessing esterases EstA, tEstA and CE, along with AcAlT and control (BL21-pET with empty plasmid) showed higher ethyl lactate production with D-lactate isomer than L-lactate, indicating superior enantio-selectivity of the enzymes for D-lactic acid. AcAlT possessing strains exhibited the least ethyl lactate production over those possessing the esterases. The image was created using Microsoft PowerPoint and Microsoft Excel.
Based on the ester titers from the preliminary screening and considering the possible interference of atmospheric oxygen in fermentation during intermittent aliquot sampling, the Phase II strains were subjected to higher cell density fermentation (OD600 = 10.0, HCD10) using a sample-wise cultivation strategy. Unlike aliquot sampling, where a small portion of the sample was removed for analysis from the same culture tube every time, sample-wise cultivation strategy employed a separate set of tubes for every sample variant and the replicate. The strategy was implemented with (Supplementary Fig. SI-4b) and without external supplementation of ethanol, and corresponding D- or L-lactic acid. Strains possessing gldA101 gene (SSL64, SSL74, SSL76, SSL79) produced more ethyl lactate than their counterparts possessing lldD gene (SSL65, SSL75, SSL77, SSL80) (Fig. 3b). The highest ethyl lactate titers were exhibited with doping by SSL74 containing CE (8.2 ± 0.4 mg/L) and SSL76 containing EstA (9.5 ± 0.4 mg/L) (Fig. 3b). A quick study conducted by analyzing the spent medium showed that despite increase in the lactate production by the Phase II strains (0.35 g/L in WT to 1.45 g/L in SSL74), there was no effect on the acetate (1.5 g/L in WT and 1.6 g/L in SSL74) produced from acetyl CoA37. This observation supported the fact that pyruvate to acetyl CoA flux was unaffected by the overexpression of lactate genes38,39. However, well-controlled reactor-scale studies were necessary to comprehend the maximum ester biosynthesis potential of these strains. Therefore, batch fermentation with 60 g/L of glucose in hybrid M9 media was performed in a bioreactor to evaluate the ability of SSL74 and SSL76 to produce the short-chain esters (ethyl lactate and ethyl acetate) under anaerobic conditions.
Although all the earlier experiments were performed by inducing the seed or pre-culture before subjecting it to the high cell density fermentation, to the best of our knowledge, there was no literature available on the effect of pre-culture induction on fermentation titers. Therefore, we studied this effect at the bioreactor scale with SSL76 strain. The seed culture was grown aerobically in a 1 L bioreactor, induced with 0.1 mM of IPTG and incubated for 18 h at 30 °C. The induced pre-culture was subjected to high cell density (with initial OD600 ~ 4 to 5) in a batch cultivation mode and was compared for ethyl lactate production with un-induced pre-culture fermentation. Batch fermentation with un-induced pre-cultures showed higher titer for short-chain esters in comparison to that with the induced pre-cultures, at all the time points (Fig. 4a). Hence, for further bioreactor fermentations, IPTG induction was used only during the production phase. Separate set of optimization studies was carried out and the optimal IPTG concentration of 0.1 mM was selected for induction under this study (Supplementary Fig. SI-6a). Detailed optimization studies are mentioned in the Supplementary information SI-6.
Batch fermentation analysis of engineered E. coli strains SSL74 and SSL76 in a 1 L bench-top bioreactor at pH 7 and under anaerobic conditions. (a) E. coli SSL74 uninduced vs induced pre-culture cultivation. Based on the results, further fermentations were carried out with uninduced pre-cultures. (b) E. coli SSL74 and E. coli SSL76 ethyl lactate and ethyl acetate concentration time curves, (c) SSL74 OD600, glucose, lactate, acetate, succinate and ethanol concentration time curves, (d) SSL76 OD600, glucose, lactate, acetate, succinate and ethanol concentration time curves. The image was created using Microsoft PowerPoint and Microsoft Excel.
Uninduced pre-cultures of SSL74 and SSL76 were subjected to high cell density batch fermentation. The kinetics of glucose, biomass, succinate, lactate, acetate, ethanol, and short-chain esters under batch cultivation mode are shown in Fig. 4b–d. Results showed that short-chain esters production peaked at 29 h (ethyl acetate (25.7 mg/L) and ethyl lactate (4.5 mg/L)) and then leveled off at later stage (Fig. 4b). Similar trend was observed for production of precursors indicating the complimentary correlation of ester production with precursors production. At 20 h of E. coli SSL74 cultivation, 12.6 g/L of lactic acid was produced under anaerobic conditions along with 14 g/L, 6.5 g/L and 5.2 g/L of ethanol, acetic acid and succinic acid, respectively. After 20 h, production of precursors leveled off (Fig. 4c). Similarly, both precursors and esters productions peaked for SSL76 at 20 h as well (Fig. 4d). Secondly, 95% and 85% glucose was consumed at 20 h while ethyl lactate and ethyl acetate were constantly produced by E. coli SSL74 and E. coli SSL76 strains, respectively (Fig. 4c,d).
To maximize the short-chain esters titers, anaerobic dual-pulse fed-batch cultivation was conducted at pH 7 by addition of 25 g/L of glucose at 6 h and 20 h. As seen in Fig. 5, dual-pulse fed-batch cultivation approach resulted in more than three-fold increase in ethyl acetate production (from 25.7 to 80 mg/L) and two-fold increase in ethyl lactate production (from 4.5 to 9 mg/L) in comparison to batch mode cultivation (Fig. 5a,b). In contrast to batch mode, we observed constant increase in ethyl acetate and ethyl lactate production beyond 20 h. Lactic acid, acetic acid, and ethanol productions were also observed to be gradually increasing suggesting that the availability of higher precursors concentration favored the esters production by engineered strains.
Fed-batch fermentation analysis of recombinant E. coli SSL74 and SSL76 strains in a 1 L bench-top bioreactor at pH 7. (a) E. coli SSL74 ethyl lactate and ethyl acetate concentration time curves, (b) E. coli SSL76 ethyl lactate and ethyl acetate concentration time curves, (c) SSL74 OD600, glucose, lactate, acetate, succinate and ethanol concentration time curves, (d) SSL76 OD600, glucose, lactate, acetate, succinate and ethanol concentration time curves. The image was created using Microsoft PowerPoint and Microsoft Excel.
As discussed earlier, pH could be one of the limiting parameters during high cell density cultivation and could significantly affect ester biosynthesis40,41. Both the strains, SSL74 and SSL76, were equally efficient in ester production (Fig. 5a,b). Hence, only SSL76 was selected as a representative strain for the further study. To analyze the effect of pH, SSL76 was used as a representative platform strain and fed-batch cultivation was conducted at pH 6. At 72 h, despite relatively lower titers of ethanol, lactate and acetate in the medium than those with pH 7.0 (Fig. 6b), and maintaining the same cell density and glucose feeding, there was two-fold increase in the ethyl lactate production (18.2 mg/L) and three-fold increase in ethyl acetate production (225 mg/L) over pH 7 was observed (Figs. 6a and 5b). This suggested that pH was a crucial factor for short-chain esters production using esterases.
Fed-batch fermentation analysis of recombinant E. coli SSL76 strains in a 1 L bench-top bioreactor at pH 6. (a) E. coli SSL76 ethyl lactate and ethyl acetate concentration time curves at pH 6, (b) SSL76 OD600, glucose, lactate, acetate, succinate and ethanol concentration time curves at pH 6. Concentrations for pH 6 experiment were corrected to the added volumes of acid and base for pH adjustment. The image was created using Microsoft PowerPoint and Microsoft Excel.
Esters are one of the major aroma components in wine and beer, produced due to the action of yeasts such as Saccharomyces cerevisiae and Brettanomyces bruxellensis1,29,42,43. B. bruxellensis is a non-conventional yeast used in the fermentation of craft and specialty beers and natural wine for its ability to produce secondary metabolites like esters during fermentation. It enhances the taste and aroma of the final product44. Like yeasts, pathogenic bacteria, e.g. Pseudomonas are also known to synthesize potent esterases involved with a rhamnolipid synthesis which exhibits an important role in their swarming motility, pathogenicity, outer membrane, and biofilm formation30,31. Hence, as a first step, mining and bioprospecting of various esterifying enzymes was performed. In total, five different esterases (EstA from P. aeruginosa along and its truncated variant tEstA, AXE2 and CE from B. bruxellensis, EEB1 from S. cereviciase), and an acyltransferase, AcAlt from Pichia pastoris were selected and considered for heterologous cloning into E. coli35,36 (Fig. 1a). Phase I strains were constructed by transforming E. coli BL21-DE3 with the plasmid constructs possessing only the esterifying enzyme gene under PT7 (Supplementary Fig. SI-2). pNPA assay, performed using the whole cell lysates of their induced biomass, exhibited the following trend of their catalytic efficiencies for ester hydrolysis; AXE2 < CE < tEstA < AcAlT < EEB1. In principle, lower catalytic efficiency for ester hydrolysis, would mean higher esterification potential. So, purportedly AXE2 must be a better esterase. However, AXE2 had a weaker affinity towards the substrate as observed from its KM value. Therefore, we investigated all the enzymes for their in vivo ester production.
As a next step, the Phase I strains were investigated for the production of ethyl lactate. First, the inherent ability of the control strain, BL21-pET to produce ethanol and lactate was analyzed. As BL21-pET produced relatively less ethanol (Supplementary Fig. SI-3a), preliminary screening of all the Phase I strains was performed with an external ethanol supplementation. Such exogenous supplementation could provide better acid:alcohol ratio, thereby driving the equilibrium to ester production41. However, they showed very low ethyl lactate titers indicating that sole external supplementation of precursors may not be sufficient. Increasing the intracellular levels of the precursors will be essential for improving the ester synthesis (Supplementary Fig. SI-3b). Hence, two distinct metabolic modules were engineered into E. coli: for precursor (alcohol and organic acid) overproduction, and for esterification (Fig. 1a), forming Phase II strains (Supplementary Fig. SI-2, Table 1). The comparative analysis of Phase II strains with intermittent aliquot sampling confirmed the in vivo esterification potential of esterases, giving significantly higher ester titers than the Phase I strains at HCD3 (Fig. 3a). Further analysis of Phase II strains was performed with sample-wise cultivation (HCD10) strategy, to avoid any oxygen interference faced during aliquot sampling. It was done with and without external doping of ethanol, to estimate whether higher precursor concentrations could drive more ester biosynthesis41. The results supported this hypothesis (Fig. 3b, Supplementary information SI-4b), indicating that increase in the precursor concentrations could improve the ester titers and it can be very well achieved at the controlled bioreactor scale. To reduce competition between cell growth and metabolite production, the fermentation was carried out in two stages; the first aerobic growth stage where the cells were grown till mid-exponential phase, and the second stage was maintained under anaerobic conditions to increase the production of esters. Results also exhibited SSL74 (possessing CE) and SSL76 (possessing EstA) as the potent candidates for short-chain ester biosynthesis (Fig. 3b). In addition, the esterases were more selective for D-lactate over L-lactate as one of the esterification substrates (Fig. 3b). Multiple parameters; IPTG concentration, phase for induction, pre-culture incubation temperature, and fermentation temperature were optimized (Supplementary information SI-6) for improving the ester production. Ultimately, to analyze the esterification potency of these best performing esterases (CE and EstA) at higher scale and under controlled environmental conditions, scale-up studies were carried out with a bench-top bioreactor in batch mode.
Initially, the effect of pre-culture induction on ester production was analyzed in the bioreactor. To the best of our knowledge, there was no literature available on this effect. For this experiment, the results showed that the uninduced pre-cultures produced higher titers of ethyl lactate with high cell density fermentation than the induced ones (Fig. 4a). The lower titers with induced pre-cultures could be associated with the preliminary metabolic burden, which may have led to decreased productivity45,46,47,48,49. Secondly, it is reported that high expression of heterologous proteins results in an adverse and unpredictable outcome on the induced cell development. In contrast, too low protein expression levels result into reduced encounter frequency between the recombinant protein and the precursors45,46,47,48,50. Hence, the bioreactor studies were carried out under optimized conditions and cultures were induced only during the production phase (Supplementary information SI-6, Fig. 4a).
Despite optimally controlled parameters in batch fermentation, ethyl lactate titers obtained with SSL74 and SSL76 were almost equivalent to the ones obtained in the shake-flasks (Fig. 4b). These titers could be attributed to the substrate limitation and/ or pH changes during the batch mode51. Therefore, for further improvement in the ester titers, anaerobic dual-pulse fed-batch cultivation was conducted at pH 7, which resulted in the two–threefold improvement in the ester titers. The results indicated that pH maintenance and glucose supplementation resulted in gradual increase in precursor concentrations, thereby improving the ester production from the engineered strains (Fig. 5a–d). However, pH could be one of the limiting parameters during high cell density cultivation and could significantly affect ester biosynthesis40,41. Difference in the pH can lead to variable ionic forms of the enzyme active site, ultimately affecting the enzyme activity52. In addition, only undissociated acids can participate in an esterification reaction, and the lower pH leads to higher fraction of such undissociated acid molecules in the system41. Hence, optimal esterification can be achieved at lower pH. On the other hand, for E. coli, it was reported that the pH values of less than 6.0 would negatively affect the cell growth52,53. Therefore, for in vivo esterification, it was necessary to select an optimal pH at which the enzyme activity would be relatively high and at the same time without negatively affecting the cell growth54. Hence, a separate set of in vitro study was performed for pH optimization. The results exhibited the highest ester production at pH 6.0 (Supplementary information SI-8). Therefore, to analyze the effect of pH 6.0 on in vivo esterification, SSL76 was used as a representative strain, and dual-pulse fed-batch cultivation was conducted at pH 6. Our results supported the earlier finding, that at pH 6, the ethyl acetate titer improved threefold, whereas the ethyl lactate titer increased by twofold over those observed under pH 7 (Fig. 6a). This suggested that controlled lower pH and dual-pulse of glucose during fed-batch cultivation were crucial factors for increasing the esterase catalyzed short-chain ester production from the engineered E. coli.
In this study, we evaluated various esterases for their esterification potential to produce short-chain esters, ethyl lactate and ethyl acetate. The fermentation parameters were optimized at the flask as well as the bioreactor scales for producing esters at higher titers. Overall, the results revealed that abundant availability of metabolic precursors ethanol, lactic acid, and acetic acid favored the short-chain esters production, while pH played a critical role in maximizing the enzyme activity for product formation. It was evident that EstA (from P. aeruginosa), and CE (from B. bruxellensis) showed comparable efficiencies in short-chain ester production. Therefore, the fed-batch fermentation by SSL76 strain (possessing EstA) overexpressing the genes for both ethanol and lactate synthesis, resulted in 225 mg/L of ethyl acetate and 18.2 mg/L of ethyl lactate at pH 6. This is the highest reported ethyl lactate titer from an engineered E. coli. Further improvement in esterification rate via esterases can be achieved through protein engineering and/or reactive extraction approaches using organic solvents. Conclusively, our study has successfully demonstrated the esterification potential of esterases, and we envision that in the future this strategy can be extended to other established and emerging model microbial hosts.
All reagents were purchased from Sigma Aldrich (St. Louis, MO), unless otherwise noted. LB and hybrid M9 medium (100 mL/L of 10X M9 salts: 67.8 g/L Na2HPO4, 30 g/L KH2PO4, 5 g/L NaCl, 10 g/L NH4Cl; 1 mL/L of 1 M MgSO4, 1 mL/L of 1 M CaCl2, 1 mL/L of 1 g/L Thiamine.HCl, 5 g/L of yeast extract, 1 mL/L of trace metal solution: 0.15 g/L H3BO4, 0.065 g/L CoSO4, 0.05 g/L ZnSO4.7H2O, 0.015 g/L MnCl2.4H2O, 0.015 g/L NaMo4.2H2O, 0.01 g/L NiCl2.6H2O, 0.005 g/L CuSO4.5H2O, 3 g/L ferric ammonium citrate) were used for the cultivation2,55,56,57. LB broth and M9 salts were purchased from BD Difco™ (Fisher scientific, AZ). Glucose was added to the final concentrations of 25–100 g/L (2.5–10%) to the medium based on experimental requirement. Working antibiotic concentrations were as follows: carbenicillin (100 µg/mL), chloramphenicol (25 µg/mL).
All the esterase and acyltransferase genes were codon optimized and synthesized from Twist Biosciences, USA. The mutated glycerol dehydrogenase gene (gldA101) encoding for D-lactate hydrogenase activity was amplified from the plasmid by pYY158. L-lactate dehydrogenase gene (lldD) was amplified from E. coli K12 (MG1655) gDNA. pACYDuet-Zm-pdc-Zm-adh plasmid possessing genes for cloning ethanol biosynthesis pathway was a gift from Dr. David Nielsen (Arizona State University, Tempe). Primary plasmid construction and cloning was performed in E. coli DH5α. For protein expression and further assays, all the plasmids were transformed in E. coli BL21-DE3 strains (Phase I strains) (Supplementary Fig. SI-2). Dual chemical transformation was performed for Phase II strains possessing both the plasmids; one possessing lactate dehydrogenase gene, esterifying enzyme gene and pct in pET15b plasmid while the other possessing ethanol biosynthesis pathway genes in pACYDuet plasmid (Supplementary Fig. SI-2). All the expression strains have been enlisted in Table 1 with their corresponding plasmid compositions. Steps involved in plasmid construction have been schematically represented in SI-2. Primers used for gene amplifications and cloning have been enlisted in Supplementary Table SI-7.
All the esterases were simultaneously screened59 using p-nitrophenyl acetate (pNPA) as a substrate for hydrolysis. Phase I strains were grown in LB medium with 100 µg/mL carbenicillin and induced using 1 mM IPTG during mid-exponential phase. The cells were harvested after 18 h of growth at 37 °C at 180 rpm. The pellets were stored at − 80 °C and lysed by in-house designed lysis strategy using SoluLyse60. Protein concentration of the cell lysate was measured using Bradford’s method60 and was used as a crude enzyme for the esterase assay. 5 mM pNPA was prepared in 1:1 ethanol–water mixture. The assay was performed using reaction concentrations of 70 µg/mL of lysate protein and 0.1 mM of pNPA in a 0.1 M Na-phosphate buffer (pH 7) at 37 °C for 1 h in a microtiter plate at a final volume of 200 µL in triplicates61,62. The absorbance was recorded at 410 nm after 30 min and the enzyme activity parameters (KM, kcat/KM) were calculated.
Seed cultures were grown overnight at 37 °C by inoculating the Phase II strain stocks in 5 mL LB medium with antibiotics. 1% (v/v) seed culture was inoculated in 200 mL LB medium in 1 L flask with appropriate antibiotics and incubated in shaker with 250 rpm at 37 °C. Once pre-culture attains the OD600 of 0.5–0.6 after 2.5–3 h, cultures were induced with 1 mM IPTG and incubated further at 37 °C for 3 h. Effect of pre-culture incubation time and temperature on ethyl lactate production was analyzed, where the pre-cultures were induced with 0.1 mM IPTG after 3 h incubation at 37 °C and incubated further at 30 °C and 37 °C for 20 h. High-cell density (HCD) culture experiments were conducted by inoculating the aerobically grown pre-cultures in hybrid M9 medium with antibiotics at different optical densities (3.0 as HCD3, 10.0 as HCD10, 20.0 as HCD20, 30.0 as HCD30), varying glucose supplementations (2%, 5%, 10% w/v), different IPTG concentrations (0.01, 0.1, 0.5, 1.0 mM), and temperatures (25 °C, 30 °C, 37 °C); as per experimental design and optimization strategy. HCD cultures were grown under low oxygen condition in triplicates in dual-lock tubes (3 mL culture volumes) which were subjected to ‘intermittent aliquot sampling’ during cultivation. These cultures were externally doped with 2 g/L (D/L) lactate and 10 g/L ethanol, and comparative studies were performed with the undoped HCD cultures for ethyl lactate production. To evaluate the effect of mode of cultivation and to avoid any oxygenation of the fermentation conditions, further experiments were performed with ‘sample-wise cultivation’ of cultures in 2 mL microcentrifuge tubes, where every sample was distinctly cultured in a separate tube, in triplicates.
Batch fermentation experiments were conducted in 1 L bench-top bioreactors with working volume of 500 mL (BioFlo/CelliGen 115, New Brunswick Scientific Inc., Enfield, CT, USA). High performing recombinant strains (SSL74 and SSL76) from the strain screening and selection experiments were selected for bioreactor fermentations. Recombinant strains of E. coli (SSL74 and SSL76) were inoculated with 100 μL of glycerol master stock culture in a shake flask with 50 ml LB medium containing 100 μg/mL carbenicillin and 20 μg/mL chloramphenicol and incubated for 18 h at 37 °C and 250 rpm. This pre-culture is used to inoculate seed culture for bioreactor fermentations at 2% v/v. Seed cultures of E. coli SSL74 and SSL76 were grown in a 1 L bioreactor in LB broth containing 100 μg/mL carbenicillin and 20 μg/mL chloramphenicol. This seed cultures were incubated for 20 h at 30 °C and 200 rpm. To achieve high cell density cultivation, seed cultures were grown aerobically by maintaining dissolved oxygen (DO) level at 40% in a gas flow cascade mode until OD600 value reached about 2.7 to 2.9. The resultant cell pellet obtained after centrifugation (Thermofischer Scientific Sorvall Legend XTR Centrifuge) (3973 rpm, 10 min, 4 °C) of the seed culture was washed and suspended in hybrid M9 medium containing 100 μg/mL carbenicillin and 20 μg/mL chloramphenicol. Hybrid M9 medium recipe is provided in supplementary materials. 0.1 mM of IPTG was added after 3 h to a bioreactor with working volume of 500 mL containing hybrid M9 medium, inoculated with high cell density culture (initial OD600 was ~ 4 to 5). The pH was maintained at appropriate value by auto-addition of 12 N NaOH and 1 N H2SO4. A dual-pulse anaerobic fed-batch fermentation experiment was also conducted in bench-top bioreactors with 500 mL as the initial working volume where an additional glucose of 25 g/L was manually fed to the bioreactor at 6 h and 20 h. The rest of the fermentation conditions were identical to the batch fermentation experiment, unless otherwise specified.
Glucose, acetic acid, lactic acid, and succinic acid analyses were performed on an Agilent 1100 HPLC equipped with an Aminex 87H column, a Diode-Array Detection (DAD) and a refractive index detector (RID). Culture broths were collected at appropriate time intervals and centrifuged (Eppendorf Centrifuge 5415 D) at 12,000 rpm and 2 min. The supernatants were collected, appropriately diluted, filtered and injected onto an ion exchange HPLC column (an Aminex 87H column). The mobile phase was 5 mM H2SO4 at a flow rate of 0.6 mL/min. The column temperature was at 70 °C and the RID detection (for glucose quantification) temperature was at 37 °C and DAD detection (for acids quantification) at 210 nm. Enzyme based D(−)/L( +) lactic acid detection kit (R-biopharm) was used to verify the optical purity of lactate from the engineered strains.
Ester were quantified using GC–MS analysis. For the analyses, analytes in the culture supernatants and/or whole cultures were extracted using chloroform in a 5:4 (v/v) ratio for 10 min at RT, on a vortex machine, in 2 mL microcentrifuge tubes. The mixture was centrifuged at 10,000 rpm for 2 min and the 1 µL of organic layer was injected into the GC system (7890A) coupled with 5975C inert MSD system from Agilent Technologies. The injection was made in a splitless mode. For GC system, hydrogen was used as a carrier gas with a flow rate of 1 mL/min and analytes were separated on DB-5ms column (30 m × 0.25 mm, 0.25 µm) from Agilent Technologies. The oven temperature was ramped with an initial temperature of 50 °C with a 1 °C/min to 58 °C. Next, a 25 °C/min ramp was deployed to 250 °C. Post-run was at 300 °C for 3 min to avoid any interference from remnants2. Analytes were detected using selected ion monitoring (SIM) mode. Dynamic SIM strategy was employed as follows: for ethyl lactate, ions 45.0, 46.0, 74.8, 74.9, 75.0, 75.1 were detected from 8.00 to 15.68 min; for ethyl acetate, ions 43.0, 60.8, 60.9, 61.0, 61.1, 71.0 were detected from 2.50 to 8.00 min. 75.0 and 61.0 were used as characteristic ions for ethyl lactate and ethyl acetate, respectively.
HCD cultures were cultivated in biological triplicates, whereas the fermentation samples were taken as technical duplicates for metabolite and ester analysis. Mean values and standard deviations were calculated by Microsoft Excel standard functions. P values used for determining statistical significance of our results were calculated in Microsoft Excel using Student’s t-test.
All data generated and analyzed during this study are included in this article and its supplementary information.
Rodriguez, G. M., Tashiro, Y. & Atsumi, S. Expanding ester biosynthesis in Escherichia coli. Nat. Chem. Biol. 10, 259–265. https://doi.org/10.1038/nchembio.1476 (2014).
Article CAS PubMed PubMed Central Google Scholar
Lee, J.-W. & Trinh, C. T. Microbial biosynthesis of lactate esters. Biotechnol. Biofuels 12, 226. https://doi.org/10.1186/s13068-019-1563-z (2019).
Article CAS PubMed PubMed Central Google Scholar
Research, G. V. U.S. Esters Market Size, Share & Trends Analysis Report by Product (Fatty Esters, Phosphate Esters, Acrylic Esters, Cellulose Esters, Allyl and Aromatic Esters), by Application, And Segment Forecasts, 2019–2025. 124 (2019).
Liu, M. et al. Transformation of alcohols to esters promoted by hydrogen bonds using oxygen as the oxidant under metal-free conditions. Sci. Adv. 4, eaas9319. https://doi.org/10.1126/sciadv.aas9319 (2018).
Article ADS CAS PubMed PubMed Central Google Scholar
Walls, L. E. & Rios-Solis, L. Sustainable production of microbial isoprenoid derived advanced biojet fuels using different generation feedstocks: A review. Front. Bioeng. Biotechnol. 8, 599560–599560. https://doi.org/10.3389/fbioe.2020.599560 (2020).
Article PubMed PubMed Central Google Scholar
Rosales-Calderon, O. & Arantes, V. A review on commercial-scale high-value products that can be produced alongside cellulosic ethanol. Biotechnol. Biofuels 12, 240. https://doi.org/10.1186/s13068-019-1529-1 (2019).
Article CAS PubMed PubMed Central Google Scholar
Lee, S., Kang, M., Bae, J.-H., Sohn, J.-H. & Sung, B. H. Bacterial valorization of lignin: strains, enzymes, conversion pathways, biosensors, and perspectives. Front. Bioeng. Biotechnol. https://doi.org/10.3389/fbioe.2019.00209 (2019).
Article PubMed PubMed Central Google Scholar
Xu, Z., Lei, P., Zhai, R., Wen, Z. & Jin, M. Recent advances in lignin valorization with bacterial cultures: Microorganisms, metabolic pathways, and bio-products. Biotechnol. Biofuels 12, 32. https://doi.org/10.1186/s13068-019-1376-0 (2019).
Article CAS PubMed Central Google Scholar
Ning, P. et al. Recent advances in the valorization of plant biomass. Biotechnol. Biofuels 14, 102. https://doi.org/10.1186/s13068-021-01949-3 (2021).
Article PubMed PubMed Central Google Scholar
Khosravanipour Mostafazadeh, A. et al. in Biomass, Biofuels, Biochemicals (eds Ashok, P., Rajeshwar, D. T., & Sunita, V.) 583–636 (Elsevier, 2021).
Lee, J.-W. & Trinh, C. T. Towards renewable flavors, fragrances, and beyond. Curr. Opin. Biotechnol. 61, 168–180. https://doi.org/10.1016/j.copbio.2019.12.017 (2020).
Article CAS PubMed Google Scholar
Panten, J. & Surburg, H. Flavors and fragrances, 1. General aspects.Ullmann's Encycl.Ind.Chem.1–9 (2000).
Kruis, A. J. et al. Microbial production of short and medium chain esters: Enzymes, pathways, and applications. Biotechnol. Adv. 37, 107407. https://doi.org/10.1016/j.biotechadv.2019.06.006 (2019).
Article CAS PubMed Google Scholar
Verstrepen, K. J. et al. Expression levels of the yeast alcohol acetyltransferase genes ATF1, Lg-ATF1, and ATF2 control the formation of a broad range of volatile esters. Appl. Environ. Microbiol. 69, 5228–5237. https://doi.org/10.1128/aem.69.9.5228-5237.2003 (2003).
Article ADS CAS PubMed PubMed Central Google Scholar
Park, Y. C., Shaffer, C. E. & Bennett, G. N. Microbial formation of esters. Appl. Microbiol. Biotechnol. 85, 13–25. https://doi.org/10.1007/s00253-009-2170-x (2009).
Article CAS PubMed Google Scholar
Zhang, Y. et al. Engineering of Serine-Deamination pathway, Entner-Doudoroff pathway and pyruvate dehydrogenase complex to improve poly(3-hydroxybutyrate) production in Escherichia coli. Microb. Cell Fact. 13, 172. https://doi.org/10.1186/s12934-014-0172-6 (2014).
Article CAS PubMed PubMed Central Google Scholar
Yin, X. et al. Metabolic engineering in the biotechnological production of organic acids in the tricarboxylic acid cycle of microorganisms: Advances and prospects. Biotechnol. Adv. 33, 830–841. https://doi.org/10.1016/j.biotechadv.2015.04.006 (2015).
Article CAS PubMed Google Scholar
Atsumi, S., Hanai, T. & Liao, J. C. Non-fermentative pathways for synthesis of branched-chain higher alcohols as biofuels. Nature 451, 86–89. https://doi.org/10.1038/nature06450 (2008).
Article ADS CAS PubMed Google Scholar
Liu, Y. et al. Biochemical characterization of a first fungal esterase from rhizomucor miehei showing high efficiency of ester synthesis. PLoS ONE 8, e77856. https://doi.org/10.1371/journal.pone.0077856 (2013).
Article ADS CAS PubMed PubMed Central Google Scholar
Nardi, M., Fiez-Vandal, C., Tailliez, P. & Monnet, V. The EstA esterase is responsible for the main capacity of Lactococcus lactis to synthesize short chain fatty acid esters in vitro. J. Appl. Microbiol. 93, 994–1002. https://doi.org/10.1046/j.1365-2672.2002.01793.x (2002).
Article CAS PubMed Google Scholar
Maester, T. C. et al. Exploring metagenomic enzymes: A novel esterase useful for short-chain ester synthesis. Catalysts 10, 1100 (2020).
Martinez, K. A. 2nd. et al. Cytoplasmic pH response to acid stress in individual cells of Escherichia coli and Bacillus subtilis observed by fluorescence ratio imaging microscopy. Appl. Environ. Microbiol. 78, 3706–3714. https://doi.org/10.1128/aem.00354-12 (2012).
Article ADS CAS PubMed PubMed Central Google Scholar
Wilks, J. C. & Slonczewski, J. L. pH of the cytoplasm and periplasm of Escherichia coli: Rapid measurement by green fluorescent protein fluorimetry. J. Bacteriol. 189, 5601–5607. https://doi.org/10.1128/JB.00615-07 (2007).
Article CAS PubMed PubMed Central Google Scholar
Szatmári, D. et al. Intracellular ion concentrations and cation-dependent remodelling of bacterial MreB assemblies. Sci. Rep. 10, 12002. https://doi.org/10.1038/s41598-020-68960-w (2020).
Article ADS CAS PubMed PubMed Central Google Scholar
Flamholz, A., Noor, E., Bar-Even, A. & Milo, R. eQuilibrator–the biochemical thermodynamics calculator. Nucleic Acids Res. 40, D770–D775. https://doi.org/10.1093/nar/gkr874 (2012).
Article CAS PubMed Google Scholar
Noor, E., Haraldsdóttir, H. S., Milo, R. & Fleming, R. M. T. Consistent estimation of gibbs energy using component contributions. PLOS Comput. Biol. 9, e1003098. https://doi.org/10.1371/journal.pcbi.1003098 (2013).
Article ADS CAS PubMed PubMed Central Google Scholar
Jankowski, M. D., Henry, C. S., Broadbelt, L. J. & Hatzimanikatis, V. Group contribution method for thermodynamic analysis of complex metabolic networks. Biophys. J. 95, 1487–1499. https://doi.org/10.1529/biophysj.107.124784 (2008).
Article ADS CAS PubMed PubMed Central Google Scholar
Holt, S., Miks, M. H., de Carvalho, B. T., Foulquié-Moreno, M. R. & Thevelein, J. M. The molecular biology of fruity and floral aromas in beer and other alcoholic beverages. FEMS Microbiol. Rev. 43, 193–222. https://doi.org/10.1093/femsre/fuy041 (2018).
Article CAS PubMed Central Google Scholar
Menoncin, M. & Bonatto, D. Molecular and biochemical aspects of Brettanomyces in brewing. J. Inst. Brew. 125, 402–411. https://doi.org/10.1002/jib.580 (2019).
Wilhelm, S., Gdynia, A., Tielen, P., Rosenau, F. & Jaeger, K.-E. The autotransporter esterase EstA of Pseudomonas aeruginosa is required for rhamnolipid production, cell motility, and biofilm formation. J. Bacteriol. 189, 6695–6703. https://doi.org/10.1128/JB.00023-07 (2007).
Article CAS PubMed PubMed Central Google Scholar
Kovacic, F. et al. A membrane-bound esterase PA2949 from Pseudomonas aeruginosa is expressed and purified from Escherichia coli. FEBS Open Bio 6, 484–493. https://doi.org/10.1002/2211-5463.12061 (2016).
Article CAS PubMed PubMed Central Google Scholar
Lestari, P., Elfrida, N., Suryani, A. & Suryadi, Y. Study on the production of bacterial cellulose from Acetobacter xylinum using agro-waste. Jordan J. Biol. Sci. 7, 75–80. https://doi.org/10.12816/0008218 (2014).
Ahn, J. H., Hwang, M. Y., Lee, K. H., Choi, C. Y. & Kim, D. M. Use of signal sequences as an in situ removable sequence element to stimulate protein synthesis in cell-free extracts. Nucleic Acids Res. 35, e21. https://doi.org/10.1093/nar/gkl917 (2007).
Article CAS PubMed Google Scholar
Kaushik, S., He, H. & Dalbey, R. E. Bacterial signal peptides- navigating the journey of proteins. Front. Physiol. https://doi.org/10.3389/fphys.2022.933153 (2022).
Article PubMed PubMed Central Google Scholar
Zhuang, S. et al. Production of medium-chain volatile flavour esters in Pichia pastoris whole-cell biocatalysts with extracellular expression of Saccharomyces cerevisiae acyl-CoA:ethanol O-acyltransferase Eht1 or Eeb1. Springerplus 4, 467. https://doi.org/10.1186/s40064-015-1195-0 (2015).
Article CAS PubMed PubMed Central Google Scholar
Saerens, S. M. G., Delvaux, F. R., Verstrepen, K. J. & Thevelein, J. M. Production and biological function of volatile esters in Saccharomyces cerevisiae. Microb. Biotechnol. 3, 165–177. https://doi.org/10.1111/j.1751-7915.2009.00106.x (2010).
Article CAS PubMed PubMed Central Google Scholar
Enjalbert, B., Millard, P., Dinclaux, M., Portais, J.-C. & Létisse, F. Acetate fluxes in Escherichia coli are determined by the thermodynamic control of the Pta-AckA pathway. Sci. Rep. 7, 42135. https://doi.org/10.1038/srep42135 (2017).
Article ADS CAS PubMed PubMed Central Google Scholar
Colletti, P. F. et al. Evaluating factors that influence microbial synthesis yields by linear regression with numerical and ordinal variables. Biotechnol. Bioeng. 108, 893–901. https://doi.org/10.1002/bit.22996 (2011).
Article CAS PubMed Google Scholar
Hua, Q., Joyce, A. R., Fong, S. S. & Palsson, B. Metabolic analysis of adaptive evolution for in silico-designed lactate-producing strains. Biotechnol. Bioeng. 95, 992–1002. https://doi.org/10.1002/bit.21073 (2006).
Article CAS PubMed Google Scholar
Ramsay, C. M. & Berry, D. R. Effect of temperature and pH on the formation of higher alcohols, fatty acids and esters in the malt whisky fermentation. Food Microbiol. 1, 117–121. https://doi.org/10.1016/0740-0020(84)90021-2 (1984).
Cui, Y., He, J., Yang, K.-L. & Zhou, K. Production of isopropyl and butyl esters by Clostridium mono-culture and co-culture. J. Ind. Microbiol. Biotechnol. 47, 543–550. https://doi.org/10.1007/s10295-020-02279-3 (2020).
Article CAS PubMed Google Scholar
Kruis, A. J. et al. Contribution of Eat1 and other alcohol acyltransferases to ester production in Saccharomyces cerevisiae. Front. Microbiol. https://doi.org/10.3389/fmicb.2018.03202 (2018).
Article PubMed PubMed Central Google Scholar
Tyrawa, C., Preiss, R., Armstrong, M. & van der Merwe, G. The temperature dependent functionality of Brettanomyces bruxellensis strains in wort fermentations. J. Inst. Brew. 125, 315–325. https://doi.org/10.1002/jib.565 (2019).
Serra Colomer, M., Funch, B. & Forster, J. The raise of Brettanomyces yeast species for beer production. Curr. Opin. Biotechnol. 56, 30–35. https://doi.org/10.1016/j.copbio.2018.07.009 (2019).
Article CAS PubMed Google Scholar
Moon, T. S., Yoon, S.-H., Lanza, A. M., Roy-Mayhew, J. D. & Prather, K. L. J. Production of glucaric acid from a synthetic pathway in recombinant Escherichia coli. Appl. Environ. Microbiol. 75, 589–595 (2009).
Article ADS CAS PubMed Google Scholar
Moon, T. S., Dueber, J. E., Shiue, E. & Prather, K. L. J. Use of modular, synthetic scaffolds for improved production of glucaric acid in engineered E. coli. Metab. Eng. 12, 298–305 (2010).
Article CAS PubMed Google Scholar
Jones, K. L., Kim, S.-W. & Keasling, J. Low-copy plasmids can perform as well as or better than high-copy plasmids for metabolic engineering of bacteria. Metab. Eng. 2, 328–338 (2000).
Article CAS PubMed Google Scholar
Couto, M. R., Rodrigues, J. L. & Rodrigues, L. R. Optimization of fermentation conditions for the production of curcumin by engineered Escherichia coli. J. R. Soc. Interface 14, 20170470. https://doi.org/10.1098/rsif.2017.0470 (2017).
Article CAS PubMed PubMed Central Google Scholar
Varman, A. M., Xiao, Y., Pakrasi, H. B. & Tang, Y. J. Metabolic engineering of Synechocystis sp. strain PCC 6803 for isobutanol production. Appl. Environ. Microbiol. 79, 908–914. https://doi.org/10.1128/AEM.02827-12 (2013).
Article ADS CAS PubMed Central Google Scholar
Donovan, R. S., Robinson, C. W. & Glick, B. Optimizing inducer and culture conditions for expression of foreign proteins under the control of the lac promoter. J. Ind. Microbiol. 16, 145–154 (1996).
Article CAS PubMed Google Scholar
Philip, P. et al. Parallel substrate supply and pH stabilization for optimal screening of E. coli with the membrane-based fed-batch shake flask. Microb. Cell Factories 17, 69. https://doi.org/10.1186/s12934-018-0917-8 (2018).
Trivedi, J., Aila, M., Sharma, C. D., Gupta, P. & Kaul, S. Clean synthesis of biolubricant range esters using novel liquid lipase enzyme in solvent free medium. Springerplus 4, 165. https://doi.org/10.1186/s40064-015-0937-3 (2015).
Article CAS PubMed PubMed Central Google Scholar
Chaudhry, N., Mukherjee, T. K., Mukherjee, T. K. & Mukherjee, T. K. Differential effects of temperature and pH on the antibiotic resistance of pathogenic and non-pathogenic strains of Escherichia coli. Int. J. Pharm. Pharm. Sci. 8, 146–149. https://doi.org/10.22159/ijpps.2016v8i9.12664 (2016).
Lin, C. et al. Optimization of culture conditions to improve the expression level of beta1–epsilon toxin of Clostridium perfringens type B in Escherichia coli. Biotechnol. Biotechnol. Equip. 30, 324–331. https://doi.org/10.1080/13102818.2015.1126201 (2016).
Trinh, C. T., Unrean, P. & Srienc, F. Minimal Escherichia coli cell for the most efficient production of ethanol from hexoses and pentoses. Appl. Environ. Microbiol. 74, 3634–3643. https://doi.org/10.1128/aem.02708-07 (2008).
Article ADS CAS PubMed PubMed Central Google Scholar
Trinh, C. T. & Srienc, F. Metabolic engineering of Escherichia coli for efficient conversion of glycerol to ethanol. Appl. Environ. Microbiol. 75, 6696–6705. https://doi.org/10.1128/aem.00670-09 (2009).
Article ADS CAS PubMed PubMed Central Google Scholar
Layton, D. S. & Trinh, C. T. Engineering modular ester fermentative pathways in Escherichia coli. Metab. Eng. 26, 77–88. https://doi.org/10.1016/j.ymben.2014.09.006 (2014).
Article CAS PubMed Google Scholar
Varman, A. M. et al. Photoautotrophic production of D-lactic acid in an engineered cyanobacterium. Microb. Cell Fact. 12, 117. https://doi.org/10.1186/1475-2859-12-117 (2013).
Sarnaik, A., Liu, A., Nielsen, D. & Varman, A. M. High-throughput screening for efficient microbial biotechnology. Curr. Opin. Biotechnol. 64, 141–150. https://doi.org/10.1016/j.copbio.2020.02.019 (2020).
Article CAS PubMed Google Scholar
Sarnaik, A. et al. Novel perspective on a conventional technique: Impact of ultra-low temperature on bacterial viability and protein extraction. PLoS ONE 16, e0251640. https://doi.org/10.1371/journal.pone.0251640 (2021).
Article CAS PubMed PubMed Central Google Scholar
Wang, X. et al. Purification and biochemical characterization of FrsA protein from Vibrio vulnificus as an esterase. PLoS ONE 14, e0215084. https://doi.org/10.1371/journal.pone.0215084 (2019).
Article CAS PubMed PubMed Central Google Scholar
Omedes, S. et al. B-esterases characterisation in the digestive tract of the common octopus and the European cuttlefish and their in vitro responses to contaminants of environmental concern. Environ. Res. 210, 112961. https://doi.org/10.1016/j.envres.2022.112961 (2022).
Article CAS PubMed Google Scholar
Chen, X., Alonso, A. P., Allen, D. K., Reed, J. L. & Shachar-Hill, Y. Synergy between (13)C-metabolic flux analysis and flux balance analysis for understanding metabolic adaptation to anaerobiosis in E. coli. Metab. Eng. 13, 38–48. https://doi.org/10.1016/j.ymben.2010.11.004 (2011).
Article CAS PubMed Google Scholar
We thank Prof. David Nielsen (Arizona State University, Tempe, AZ, USA) for providing us with the pACYDuet-Zm-pdc-Zm-adh plasmid. We acknowledge Mark Nguyen, Francisco Gonzalez (Arizona State University, Tempe) for their time and efforts during sequence curation and analysis. We also thank Dylan Smith (Arizona State University, Tempe) for his efforts while optimizing the in vitro esterase activity assay.
AMV acknowledges start-up funds from the School for Engineering of Matter, Transport and Energy at Arizona State University. This research was also supported in parts by Sandia National Laboratories through the awards #2184871 and #1871463 to AMV. This research was also supported in parts by the BioEnergy Technology Office (BETO), U.S. Department of Energy, through support of RWD, under agreement #26336.
These authors contributed equally: Aditya P. Sarnaik and Somnath Shinde.
Chemical Engineering Program, School for Engineering of Matter, Transport and Energy, Arizona State University, Tempe, AZ, USA
Aditya P. Sarnaik, Apurv Mhatre, Abigail Jansen, Amit Kumar Jha, Haley McKeown & Arul M. Varman
Bioresource and Environmental Security, Sandia National Laboratories, Livermore, CA, USA
Somnath Shinde, Amit Kumar Jha & Ryan Davis
You can also search for this author in PubMed Google Scholar
You can also search for this author in PubMed Google Scholar
You can also search for this author in PubMed Google Scholar
You can also search for this author in PubMed Google Scholar
You can also search for this author in PubMed Google Scholar
You can also search for this author in PubMed Google Scholar
You can also search for this author in PubMed Google Scholar
You can also search for this author in PubMed Google Scholar
A.M.V. and R.D. conceived, designed, and supervised the study. A.P.S. conducted protein engineering, strain development, lab-scale fermentation optimizations and data analysis. S.S. conducted fermentations at the reactor scale and analyzed those samples on H.P.L.C. A.M. conducted HPLC analysis. A.J. performed preliminary strain analysis and lab-scale fermentations. A.K.J. was involved with protein mining. A.P.S. and S.S. have written the manuscript, created the figures and made the plots included in the manuscript. A.P.S., S.S., A.M., A.J., A.K.J., H.M., A.M.V. and R.D. were involved with reviewing the manuscript.
Correspondence to Ryan Davis or Arul M. Varman.
The authors declare no competing interests.
Springer Nature remains neutral with regard to jurisdictional claims in published maps and institutional affiliations.
Open Access This article is licensed under a Creative Commons Attribution 4.0 International License, which permits use, sharing, adaptation, distribution and reproduction in any medium or format, as long as you give appropriate credit to the original author(s) and the source, provide a link to the Creative Commons licence, and indicate if changes were made. The images or other third party material in this article are included in the article's Creative Commons licence, unless indicated otherwise in a credit line to the material. If material is not included in the article's Creative Commons licence and your intended use is not permitted by statutory regulation or exceeds the permitted use, you will need to obtain permission directly from the copyright holder. To view a copy of this licence, visit http://creativecommons.org/licenses/by/4.0/.
Sarnaik, A.P., Shinde, S., Mhatre, A. et al. Unravelling the hidden power of esterases for biomanufacturing of short-chain esters. Sci Rep 13, 10766 (2023). https://doi.org/10.1038/s41598-023-37542-x
DOI: https://doi.org/10.1038/s41598-023-37542-x
Anyone you share the following link with will be able to read this content:
Sorry, a shareable link is not currently available for this article.
Provided by the Springer Nature SharedIt content-sharing initiative
By submitting a comment you agree to abide by our Terms and Community Guidelines. If you find something abusive or that does not comply with our terms or guidelines please flag it as inappropriate.
Scientific Reports (Sci Rep) ISSN 2045-2322 (online)

THIOPROLINE Sign up for the Nature Briefing: Translational Research newsletter — top stories in biotechnology, drug discovery and pharma.